Aerodynamics
Boundary layer flows
Numerical investigation of coherent structures in fully developed turbulent pipe flow
It has been almost a century since the significance of wall bounded turbulence has been realized and in the meantime it has remained an attractive topic for both experimental and numerical studies. Among canonical forms of wall turbulence, pipe flow has received special attention due to its industrial and scientific relevance. In this regard the goal of reducing energy losses finds even more grounds, as dissipation plays a decisive role in causing energy losses while dealing with turbulent flows. This fact, together with the increasing interest in physics of turbulent pipe flow at high Reynolds numbers have motivated construction of experimental facilities, enabling measurements at highly turbulent regimes. The same motivation has triggered countless numerical studies in terms of Direct Numerical Simulation (DNS) and Large Eddy Simulation (LES).
Recent numerical studies by Wu et al. (2012) and El Khoury et al. (2013) are among the prominent works that while extending the Reynolds number limits, have dedicated their attention to statistical flow properties and turbulent coherent structures. In a commonly accepted classification, large scale coherent structures are categorized into two main groups. Large-scale motions (LSM) are believed to be formed by Hairpin structures traveling at the same convective velocity. They are associated with occurrences of bulges of turbulent fluid at the edge of wall layer and are observed to have streamwise scale of 2-3 pipe radii and spanwise length scale of 1-1.5 radii. On the other hand very large-scale motions (VLSM) are interpreted as joint series of vorticity packets with streamwise length scale of 8-20 pipe radii (Vallikivi et al. 2015). In spite of vast range of experimental and numerical data available, many of the fundamental questions concerning LSM and VLSM are still unanswered. Such uncertainties include a uniform scaling law for their identification as well as a clear understanding of their origin and evolution mechanism. The differing views question the dependence of low wave number VLSM on geometry and outer layer variables.
The present research aims to shed light on a number of such controversies in various ranges of Reynolds numbers. In the first phase of this study, LES has been performed for turbulent viscous flow in a pipe at four different regimes ranging from bulk Reynolds number of Reb= 5,300 to Reb=37,700 (Fig. 1). Stream wise periodic length of 25 R is selected for a pipe of radius R. Mean flow features and stream wise turbulence intensities are compared with DNS data by El Khory et al. to validate the results. As depicted in Figure 1, a more distinguished logarithmic behavior becomes observable only at higher Reynolds numbers. Previous experimental and numerical studies have confirmed that a similar trend is also observable in the streamwise turbulent intensity profile. At such regimes, it is the logarithmic region that contributes most to the bulk turbulent kinetic energy production. At the same Reynolds number range, a better scale separation starts to appear between the energy containing structures with lower wave numbers and the small scale structures in the dissipation range, thereby providing a better possibility to capture large scale coherent structures. Vorticity iso-surfaces at different wall normal distances (presented in Figure 2 for two Reynolds numbers), illustrate areas of higher and lower vorticity, forming into structures rising from the wall, evolving into the mean flow and then breaking into smaller structures.
In the second phase of this study, within a collaboration with Institute of Fluid Mechanics and Technical Acoustics (ISTA) at TU Berlin, DNS of turbulent pipe flow are to be performed at a range of bulk Reynolds numbers starting from Reb=4×103 to ranges comparable with the experimental results from CoLa-Pipe (Cottbus Large Pipe). Building on the previous work by Ghosh, Sesterhenn & Friedrich (2006) and taking the effect of compressibility into account, this study also looks into the effect of temperature changes in the wall on turbulent structures. The investigations are aimed at numerical study of recent areas of focus including scaling and identification of LSMs and VLSMs. furthermore, effect of wall temperature changes in all the mentioned flow features and structures are to be clarified. Simulation of various thermal wall boundary conditions (adiabatic, isothermal) and their influence on the size and interaction of structures are some of the goals of this study.
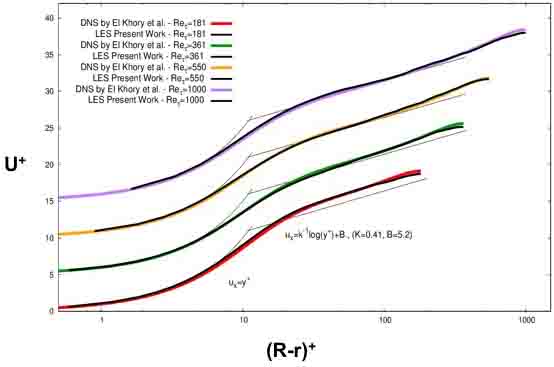
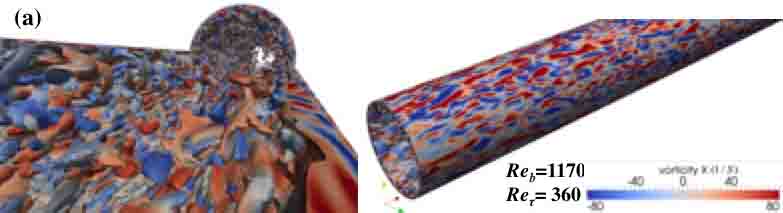
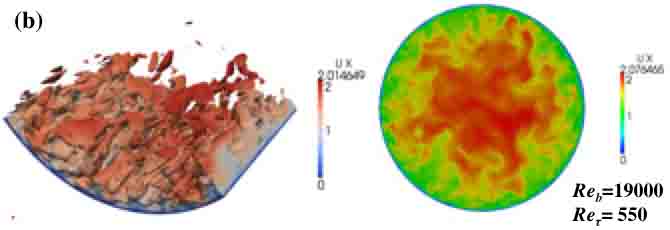
It can be observed that the sizes of these coherent structures can reach even couple of meters along the pipe axis. This phenomenon is investigated with Particle Image Velocimetry (PIV) as a non-intrusive measurement technique in CoLa-Pipe (in collaboration with LaVision GmbH) to validate and compare the results of Hot-Wire Anemometry (HWA) which have been obtained at LAS in previous studies.
As being a high Reynolds number pipe facility, for CoLa-Pipe slightly different PIV setups are necessary in comparison the other pipe facilities. Considering the relationship between the Re-number and the length of structures, large laser planes are expected in axial streamwise direction. This step is mandatory for capturing turbulent properties at high velocity ranges. Calibration of the PIV and as well as optimization of the measurement parameters such as spatial resolution, time separation between images, and number of samples should also be done.
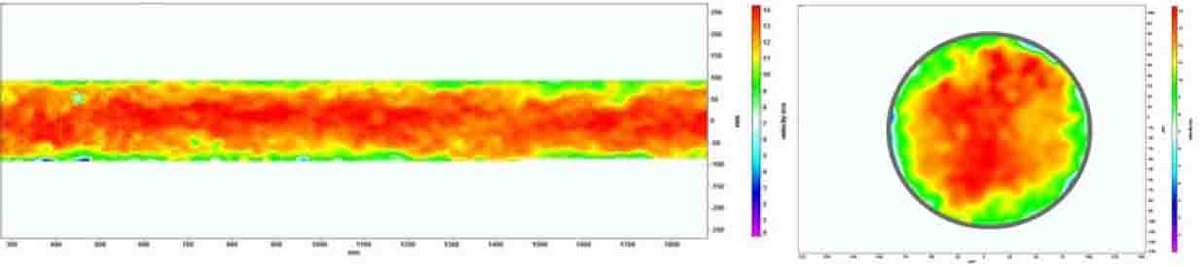
Obtaining turbulent structures at high Reynolds numbers optically with PIV requires long laser plane setups in axial direction. First of all a 2D laser plane is applied in streamwise direction to enable the identification of axial extensions of turbulent structures. In this step spatial auto-correlation is performed using streamwise velocity. The results show that after traveling a certain distance the turbulent structures show a specific correlation in terms of lengths.
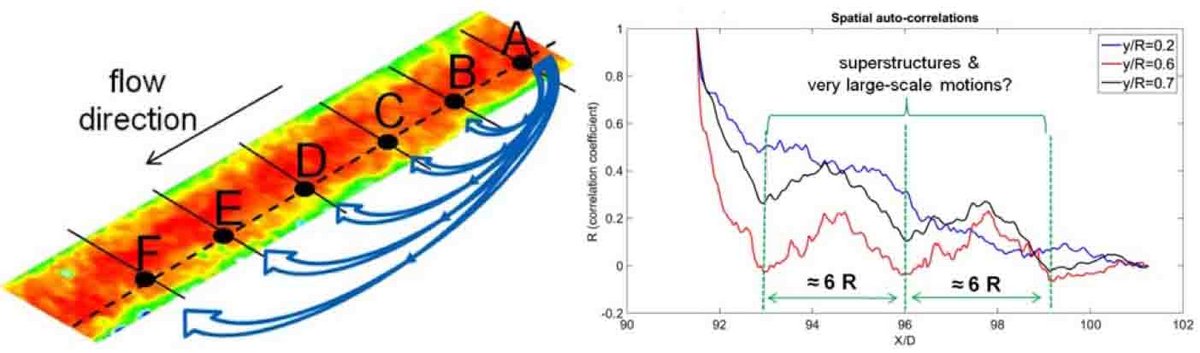
Their periodic properties can be observed at higher wall-normal distances and they show a similar behavior to previous HWA-results in CoLa-Pipe as mentioned in Öngüner et al. (2016).
Bibliography
- Adrian, R.J., 1991, Particle imaging techniques for experimental fluid mechanics, Ann. Rev. Fluid Mech. 23, 261-304
- Guala, M., Hommena, S.E., Adrian, R.J.; 2006, Large-scale and very large-scale motions in turbulent pipe flow, J. Fluid Mech., 554, 521-542
- König, F., Zanoun, E.-S., Öngüner, E., Egbers, Ch., 2014, The CoLaPipe - The New Cottbus large pipe test facility at Brandenburg University of Technology Cottbus-Senftenberg, Review of Scientific Intstruments 85, 075115
- Vallikivi, M., 2014, Wall-bounded turbulence at high Reynolds numbers, PhD thesis, Princeton University
Bibliography
- Ghosh, S., Sesterhenn, J., Friedrich, R.,"DNS and LES compressible turbulent pipe flow with isothermall wall", Direct and Large-Eddy Simulation VI, 712-728, 2006
- El Khoury, G. K., Schlatter, P., Noorani, A., Fischer, P. F., Brethouwer, G., Johansson, A. V,"Direct Numerical Simulation of Turbulent Pipe Flow at Moderately High Reynolds Numbers", Flow Turbulence Combust. 91, 475-495, 2013
- Vallikivi, M., Ganapathisubramani, B., Smits, A.,"Spectral scaling in boundary layers and pipes at very high Reynolds numbers", J. Fluid. Mech. 771, 303-326, 2015
- Wu, X., Baltzer, J. R., Adrian R. J.,"Direct numerical simulation of a 30R long turbulent pipe flow at Reτ = 685: large- and very large-scale motions", J. Fluid Mech., vol. 698, pp. 235-281, 2012